How to Build a Satellite
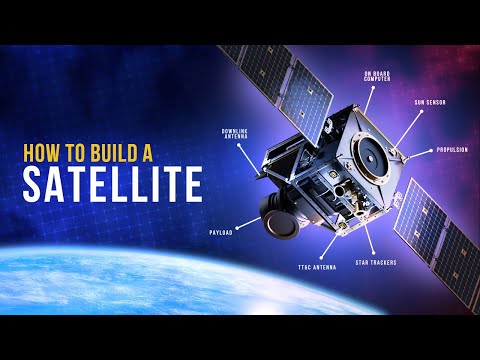
Satellites are essential tools in our modern world. They facilitate seamless global communication, gather valuable scientific data, and even allow us to explore the cosmos and other planets in the solar system. These orbiting spacecraft are true marvels of engineering. Not only must they survive extreme forces and vibrations during launch, they also need to operate reliably in the harsh environment of space. In this video we'll dive into the fascinating world of satellite technology, and the clever engineering used to ensure the success of each mission. Any satellite can be thought of as two separate systems, the payload, and the
bus. The payload is the equipment used to carry out the mission the satellite has been launched for. An earth observation mission could have a payload consisting of cameras and radar equipment, a communications mission would likely be equipped with transponders and high gain antennas, and a scientific research mission might carry various probes and sensors. The satellite bus
is essentially everything else. It includes the structure and all of the systems needed to operate the satellite and support the payload. Satellites come in a wide range of shapes and sizes. This one has a mass of just under 100 kilograms, which places it in the microsatellite category. Large satellites can weigh well over a thousand kilograms. And those in the picosatellite category can weigh less than one kilogram. One notable format in the nanosatellite category is
the CubeSat. Developed around the concept of a standardised cube-shaped unit, called 1U, CubeSats are designed to be modular and low-cost. They can scale up from 1U, with 3U, 6U and 12U being common sizes. Although they were initially developed for educational purposes, CubeSats have evolved dramatically, and are now used for all sorts of applications including state-of-the-art scientific missions and technology demonstrations. Regardless of the size of the satellite, the bus
will usually have the same seven fundamental subsystems - the mechanical structure, the on-board computer, the electrical power system, the attitude determination and control system, the propulsion system, the communications system, and the thermal control system. Let's start with the mechanical structure. It needs to be strong and stiff to survive launch, but also as light as possible to save on launch costs. Engineers work hard to reduce mass through smart design, thorough analysis, and careful material selection. As a result satellites use materials that have good stiffness and strength properties relative to their weight, like Aluminum alloys, and carbon-fibre reinforced polymers. Honeycomb composite panels, made of a lightweight core
sandwiched between thin Aluminum alloy or carbon fibre faces, provide surfaces for mounting equipment. One important factor when selecting materials for space applications is outgassing, where the vacuum of space causes materials to gradually release gases trapped within them, which can then condense on sensitive instruments, potentially endangering the mission. Metals like aluminum tend not to outgas significantly, but all materials used on the spacecraft, from big structural parts all the way down to the adhesives used to strap fasteners, need to be checked for outgassing. A process called bake-out can be used to reduce risk, where the flight hardware is heated under vacuum conditions to accelerate outgassing before it's integrated into the satellite. The satellite structure includes a separation system that attaches to the launch vehicle adapter. The total volume of the satellite is constrained by the space allocated to it in the fairing of the launch vehicle. Deployable mechanisms are often used for solar panels,
instrument booms and antennas to get around this limited envelope, with the trade -off that this introduces new possible points of failure. At the core of every satellite is the on-board computer, the brain that controls and coordinates all of the satellite's functions, including processing data, monitoring the health of the satellite through various sensors, and issuing commands to instruments and systems. The hardware is typically built around several printed circuit boards, housed in an Aluminum alloy enclosure. One major challenge with putting electronics in space is their exposure to cosmic radiation, high-energy particles that can penetrate the structure of the satellite, disrupt circuits and in the worst case lead to system failure. The on-board computer, dense with electronic components, is particularly vulnerable. Although radiation levels are
relatively low in low earth orbit, satellites venturing into higher orbits, particularly those that pass through the Van Allen radiation belts, are at significantly higher risk. To mitigate this engineers often use radiation-hardened components and shield sensitive parts—or even the whole device—with layers of thick aluminum. One of the roles of the on-board computer is to manage the electrical power system that generates, stores and distributes the power needed to operate the satellite. A one square metre area directly facing the sun just above Earth's atmosphere will
receive 1.3kW of solar power, so it's no surprise that by far the most common method of generating power for satellites is the use of solar arrays. They can be mounted to the body of the satellite, but in many cases deployable panels are used to maximise the generated power while allowing the satellite to fit within the fairing of the launcher. Some satellites even use articulated solar arrays that can be pointed directly at the sun. Most modern satellites use multi-junction
solar cells, constructed from multiple layers of different semiconductor materials. These cells are able to capture a wider range of wavelengths than less expensive single-junction cells, making them more efficient. While orbiting the Earth, satellites experience periods of eclipse when in Earth's shadow, where the solar arrays are unable to generate any power. As a result satellites need to carry batteries that are charged by the solar panels during periods of exposure to sunlight, and discharged to provide power during the eclipse. The electrical power system is controlled by
the satellite's power control unit, which in turn interfaces with the on-board computer. It monitors the charge level of the batteries, controls charging and discharging, and regulates voltage to ensure a stable power supply for all satellite systems. Next is the Attitude Determination and Control system. It's used to determine and adjust how the satellite is oriented relative
to a reference frame. This orientation is called the satellite attitude. Satellites are constantly monitoring and adjusting their attitude. This is crucial immediately after separation from the launch vehicle, when they may experience tumbling, and the ground station needs to regain control.
But it's also important for normal operation. In a single orbit a satellite might need to point the payload at a specific location, point solar panels to face the sun, and point an antenna towards a ground station. The attitude determination and control system is made up of sensors, used to determine the current satellite attitude, and actuators, used to make the necessary attitude adjustments. The strategy used for attitude determination is selected based on the pointing requirements of the mission and the size of the satellite, but normally makes use of several different sensor types, because each one has its own limitations. Satellites often carry an Inertial Measurement Unit that contains gyroscopes and accelerometers. The three gyroscopes in the IMU provide a continuous measure of any changes in satellite orientation. But this isn't enough
to obtain good pointing knowledge because gyroscopes only provide a relative measurement, and they can drift over time, resulting in an error in the predicted attitude. A common approach for precise attitude determination is to combine gyroscope measurements with data from star trackers. Star trackers provide highly accurate reference measurements of the satellite attitude that can be used to periodically correct the continuous attitude measurement provided by the gyroscopes. Depending on the mission requirements, star trackers might operate anywhere from every 10 seconds to every 20 minutes. A star tracker is a camera that captures an image of the sky, and uses an algorithm to identify bright stars. Their position in the image is compared
with a catalogue of known stars, allowing the orientation of the satellite to be determined. Star trackers can be very accurate, but may not work well if they're pointed close to the sun, or if the satellite is spinning too fast relative to the camera shutter speed, because the captured image will be blurry. And options for accommodating them on the satellite are limited because they need to have a clear field of view, and can be affected by thermal distortion. This all needs to be taken into account by the engineers designing the system. Ultimately attitude sensors are selected based on the requirements of the mission. A satellite with less stringent pointing requirements might avoid star trackers and make use of sun sensors or magnetometers instead. Sun sensors are simple devices that use photodetector cells to estimate
the attitude of the satellite relative to the sun. But they don't work during the eclipse portion of an orbit. And magnetometers, sometimes included in the IMU, measure the strength and direction of the magnetic field surrounding the satellite. These measurements are compared with a model of the expected magnetic field of the Earth, given the satellite's position, to estimate its attitude. That covers attitude determination, but what about attitude control? Most modern satellites use three-axis stabilisation, an approach to attitude control where actuators are used to precisely control satellite orientation around three axes. One common actuator is the reaction wheel, which consists of a flywheel attached to an electric motor. Using the motor to change the
rotational velocity of the reaction wheel causes the satellite to rotate about its centre of mass in the opposite direction. A minimum of three reaction wheels mounted in orthogonal planes give the satellite full three-axis control, allowing minute adjustments to be made to the satellite attitude. One problem with reaction wheels is that, after a certain number of attitude adjustments, the incremental increases in the rotational velocity of the flywheel can bring it close to its maximum allowable rotational speed. This is called saturation, and it means that
reaction wheels cannot be used as the only method for attitude control. Magnetorquers are often used alongside reaction wheels to control attitude, and also provide a way of desaturating the reaction wheels. A magnetorquer is essentially a coil of a conductive wire wound around a magnetic core. Passing current through the coil creates a magnetic field, and when this field interacts with Earth's magnetic field a torque is generated. This torque can be used to control the satellite attitude, or if actuated at the same time as the reaction wheels it can be used to slow them down. Magnetorquers are very reliable because they have no moving parts.
But they only work in relatively low orbits where the Earth's magnetic field is sufficiently strong. Another option is simply to control attitude by using the propulsion system to fire thrusters. The propulsion system plays a crucial role in making adjustments to a satellite's orbit. This could be to move the satellite to a new orbit, or for station-keeping - corrective manoeuvres performed to maintain an existing orbit. But it can also be used for attitude control.
Common methods for propulsion are cold gas propulsion, chemical propulsion, and electric propulsion. All work using the same principle - thrust is generated by accelerating mass through a nozzle. The simplest form of propulsion is the cold gas thruster, which is the controlled expansion of a stored pressurised gas through thruster nozzles. The propellant is usually
an inert gas like nitrogen or helium. Valves, pressure transducers and pressure regulators are used to monitor system pressure and control the propellant to each of the thrusters. Higher thrust can be generated with chemical propulsion, which uses a controlled chemical reaction. In a
monopropellant system the propellant is a liquid, which decomposes into a hot gas when it comes into contact with a catalyst. A pressurant, an inert gas stored in a separate tank, supplies the pressure that forces the propellant from the tank to the thrusters. A bipropellant system uses two propellants, a fuel and an oxidiser, which are mixed and ignited in a combustion chamber, producing exhaust gases that are expelled through the nozzle to generate thrust. Electric propulsion
uses electrical energy to generate thrust. These systems work by ionizing a propellant—often a gas like xenon—and then using electric and magnetic fields to accelerate the ions to high velocities before expelling them through a nozzle. The propulsion system is selected based on the thrust requirements and the mass of the satellite. Bipropellant systems are complex but
offer high thrust, useful for large satellites. Monopropellant systems have lower thrust but simpler system design. Electric propulsion has lower thrust than the chemical propulsion options, but is very efficient, requiring less propellant. And cold gas thrusters offer low thrust but very simple design and precise control, making them a good choice for attitude control systems. As the only way for the satellite to exchange information with Earth, the communications system is of vital importance. It has two separate capabilities - Downlink, and Telemetry, Tracking and Command. The downlink system is used to beam the data generated by the payload
down to earth. The satellite uses its attitude control system to point a downlink antenna towards a ground station, and start transmitting data. The exact way this is done depends on the satellite - some transmit data once per orbit for each pass over the same ground station, others transmit to multiple ground stations, and those in geosynchronous orbits can use the same ground station continuously. Satellites transmit information to ground stations using
electromagnetic waves. These are characterised by their wavelength and frequency. Visible light, X-rays, and high energy gamma rays are all just electromagnetic waves with different frequencies. Satellite communications use electromagnetic waves in the radio frequency, or RF, part of the spectrum, mostly between 1 and 40 GHz. For convenience this part of the spectrum is split into bands with designated names. As the frequency increases the required power increases, but the data rates are also higher. And higher frequencies are more susceptible to degradation
by atmospheric attenuation. The data transmitted from a satellite is essentially a stream of bits, which can have a value of either 1 or 0. To transmit this information over vast distances using electromagnetic waves, the data needs to be encoded onto a carrier signal - a continuous sinusoidal electromagnetic wave that has a frequency in one of the frequency bands we just discussed. This is done by changing certain properties of the carrier wave, in a process called modulation. Amplitude Modulation encodes the data to be transmitted by changing the amplitude of the carrier wave. Frequency Modulation changes the frequency of
the carrier wave instead. And Phase Shift Keying adjusts the phase of the carrier wave. Most high rate communication links use one of the several forms of Phase Shift Keying modulation, which provide high data throughput and low error rates. The modulated signal is generated on board the satellite by the transmitter hardware. It's then routed through a series of switches and filters, before arriving at the downlink antenna for transmission to the ground station. Once received by the ground station the signal is demodulated and processed to obtain the valuable payload data.
The other key element of the communications system is the Telemetry, Tracking and Command subsystem, which consists of a unit called the transceiver, and various filters, switches and antennas. It uses some really clever engineering to fulfil its three different functions. The Command function is what allows the operations team to control the satellite. Commands are transmitted from
the ground station, demodulated by the TT&C hardware on the satellite, and then routed to the on-board computer for implementation. The Telemetry function transmits housekeeping data from various sensors on the satellite down to the ground station, like the temperature of critical components, the power levels in the batteries, or propellant levels in the tanks. Finally there's the Tracking function, that provides information about the position and speed of the satellite. The ground station sends a signal to the satellite, which the transceiver receives and sends back. The turnaround time provides an estimate of the distance between the satellite and the ground station. And the Doppler effect frequency shift provides an estimate of the velocity
of the satellite. These two valuable pieces of information help the operations team monitor the position and trajectory of the satellite. Although not an option for all orbits, many satellites also carry GPS receivers to enhance tracking capabilities, allowing for more effective monitoring of position and trajectory. Antennas, used to transmit and receive RF signals, are a fundamental part of any communications system. They come in many different shapes
and sizes. To compare their performance it's useful to think about a theoretical antenna, called an isotropic antenna, that radiates power uniformly in all directions. Two points located the same distance from a transmitting isotropic antenna will receive the same signal strength, regardless of where they're located around it. In reality, antennas neither radiate nor receive power uniformly across all directions, and in most cases it's not actually desirable to do so. Instead, real antennas are engineered to focus or receive energy in specific directions. This directionality can be visualised as a radiation pattern around the antenna, and is quantified by a parameter called gain. Gain is a measure of how much an antenna focuses
energy in a particular direction, relative to an isotropic antenna. An isotropic antenna has a gain of 1 in all directions, but a real antenna will have a gain greater than 1 in some directions, and less than 1 in others. The total power emitted or received is the same, it's just focused in different directions. The antennas on a satellite are selected to optimise performance
for a specific task. Downlink systems use high gain antennas which focus the transmitted signal into a narrow beam, enabling high data transfer rates. Antenna pointing mechanisms or the attitude control system can be used to direct this beam towards the ground station. TT&C systems on the other hand prioritise reliable communications instead of high data rates, because they only transmit small packets of data. They use low gain antennas with wider coverage to make sure the satellite can communicate with the ground station under all conditions, even if it's tumbling or its attitude control system isn't working properly. As a satellite zips around the Earth it's exposed to impressive extremes in temperature. Surfaces that face direct sunlight can get extremely hot,
especially with the lack of a protective atmosphere, with temperatures suddenly plummeting as the satellite enters Earth's shadow. Add the fact that many of the electronics on board will be dissipating power, locally increasing temperatures, and it's clear that controlling the satellite temperature is a huge challenge. Good thermal control is mission-critical - some parts of the payload may need to be kept within narrow temperature ranges to function as intended, and batteries or electrical components that see temperatures outside of their allowable limits are at risk of failure. Thermal control systems make use of many different technologies,
and some clever engineering, to maintain stable temperatures. Because there's no heat transfer by convection in the vacuum of space, the only way for the satellite to exchange thermal energy with the environment is by radiation. Radiators, large surfaces with high emissivity coatings, are used to radiate heat from the satellite. Heat pipes are used to transport thermal energy from hot to cold areas. Thermostat-controlled electric heaters switch on at low temperatures to make sure certain components don't get too cold. Multi-layer insulation blankets help control temperature by reflecting solar radiation when the satellite is in sunlight, and reducing radiative heat losses when the satellite is in shadow. Then there's special paints and coatings,
and phase change materials that absorb or release thermal energy by undergoing a phase change. Even the attitude control system can contribute to thermal control by changing the direction radiators and other surfaces are facing. All of these components need to work in harmony, perfectly balancing absorbing, retaining and dissipating heat, to make sure the temperature of the satellite stays within acceptable ranges. Space is hard - the harsh, isolated environment, cost of launch, and technical barriers present some formidable challenges, that can only be overcome with clever engineering and extensive testing, backed by decades of research. Whether
you're designing hardware destined for orbit, or working on an experimental project in your garage, having access to the right design tools is an important part of getting the job done. And that's why I'd like to introduce you to this video's sponsor, Onshape, a really powerful cloud-based CAD platform that makes it quick and easy to bring your designs to life. With its user-friendly interface and intuitive set of tools, Onshape makes modelling parts, building assemblies, and producing drawings easy. And it all runs in your web browser. Working with OnShape will
redefine what you come to expect from a CAD platform - it really does feel like the future of mechanical design. It has detailed version tracking, easy to use standard part libraries, and collaborative tools that allow multiple people to work on the same assembly at the same time. And you can access your files from anywhere just by logging in. There are even Android and iOS apps.
One thing I love about Onshape is the emphasis it places on community. It gives you access to public files from other users, which is a great way to learn - you can pick apart other people's models, like this detailed model of the Oresat satellite developed by a team at Portland State University, to understand the design process and how everything fits together. And if you're not sure where to start, there's loads of useful training material to help you get up to speed. So why not give it a try - it's completely free. Just head over to Onshape.pro/EfficientEngineer to create your free account and start exploring. And if you're interested in Onshape Professional,
that has even more advanced features like simulation, use the link to get 6 months for free. Thanks for watching!
2024-05-23 18:01