"The Future of Energy Storage" webinar: Electrochemical battery technology
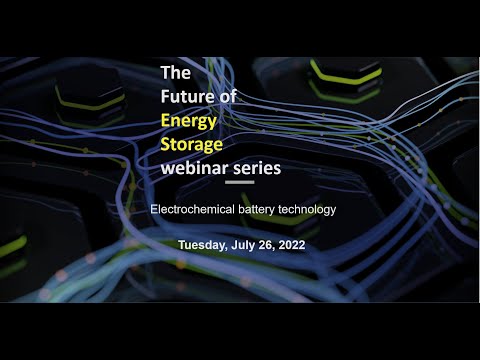
Robert Armstrong: Welcome to this first study of a series of webinars looking at the future of energy storage. My name is Bob Armstrong, I'm director of the MIT Energy Initiative. It's a pleasure to have this chance to talk to you a little bit about what we've done on a recent study that we undertook at MIT looking at the future of energy storage. This is an interdisciplinary study at the MIT bringing together faculty and senior researchers and graduate students who cover different aspects of energy storage. If you move to the next slide, Yet. This is the ninth in a series of this future of studies, which have the goal of looking at the key technologies that can play an order of one role in helping us deal with climate change That is, technologies that will be important for us to meet energy needs mid-century, while at the same time eliminating emissions from the energy system. This is particularly critical now as we realize that climate change is upon us and that we need to move very fast now if we're to meet objectives that we have. A
key part of meeting climate change and mitigating climate change is understood to be decarbonizing the electricity sector by taking advantage of renewable energy resources like solar and wind. As we use those resources in the electricity sector, we need to understand what it will take to develop that system in a way that's both reliable and affordable. Affordability is key, of course, because, in addition to decarbonizing the electricity sector, we want, at the same time, to take that decarbonized electricity and use that to help us decarbonize other parts of the economy. Of course, an interesting challenge in moving to an electricity system that's highly dependent on variable renewable resources, solar and wind, is that we need to have storage available in order that supply and demand can always be kept in match, which is a critical part of the electricity sector. Key question for the study is, what kinds of electricity storage technologies kind of policies would make it possible for us to have this VRE-dominated electricity system of the future that's affordable and reliable? All right. Next slide shows the three main messages of the study.
Those are, first of all, in the technology area. What kind of federal R&D policies can we put in place that will focus on long-duration storage technologies to support this affordable, reliable electricity system of the future? Our second main message is that we can make net-zero electricity systems affordable by tailoring storage and mix with variable renewable resources tailored to different parts of the country or different parts of the world. Then, thirdly, we're going to need to take a relook at market designs and regulatory policies and reform those to enable equitable and efficient decarbonization. Those three messages capture the three main components of the study. Technology in the first part of the study, systems analysis in the second part of the study, and then the third part looked at economics and regulatory issues. We're going to parallel that set of main messages in the webinars beginning in this hour by looking first at technologies. We'll actually spend a couple of the hours of
the webinar, two hours today and again tomorrow looking at technologies that we think can play a role in providing storage for the electricity system of the future. Then we'll look at some of the systems analysis results and then finally turn to economics and policy changes needed. With that brief introduction, I'm going to turn it over to the first two speakers this morning in this first hour of the webinar.
Really pleased to have with us Professors Yet-Ming Chiang from Materials Science and Engineering and Fikile Brushett from the Department of Chemical Engineering. They were two members of a very talented team looking at the electrochemistry approaches to storage in the electricity system. Yet, let me turn it to you. Yet-Ming Chiang: Thank you, Bob. Let's see. Here we go. The message that Bob called out is this first one, federal R&D policy should focus on long-duration storage technologies to support affordable, reliable future electricity systems. What I want to do in the coming
slides along with Fikile Brushett is to explain how we arrive at that takeaway. It involved a consideration of a broad range of electrochemical storage technologies. Our approach was to take a look first at how much storage, grid, and total storage might be needed globally by 2030 in near-term, 2050 in the longer term. Based on that estimated total storage, first what are the materials and supply chain constraints? We're not going to address that in this hour, but Elsa Olivetti and Bob Jaffe will address in the following presentation. What our group did was to look at electrochemical storage technologies that could be solutions and to consider what the manufacturing costs and other constraints and as well as opportunities are in arriving at the recommendations that we present in this study. We will be capturing really just a snapshot of the study itself. Our results here
are represented in a chapter, which is available for all of the viewers and readers. The study group for us is the group in the box on the right-hand side here. Bob was the chair of the study, I was a co-chair for this group. The study group was led by Fik and I. You see the other participants here. I especially want to point out or call out the contributions of our student members here. Well, actually, three of whom have
now graduated and what you see listed here are their current affiliations and one Sasha who is shortly to graduate. Each of these students had a significant role in the writing of particular sections of the electrochemical chapter. Now, which electrochemical storage systems did we consider? There are enormous diversity of battery chemistries out there.
It's a certainty that, for instance, your favorite battery chemistry may not be considered in detail in our chapter because of the constraints that we placed on the system to be considered number one, TRL 6 or greater. You see at the bottom definition of TRL 6 that the technology has been demonstrated in some relevant environment. This means that a full-scale battery has been built and tested. There are many developing chemistries all the time historically and today in the battery field, many of which are promising, but we do not consider in the study because of this constraint that we placed on ourselves. The three main categories we'll talk about today are lithium-ion batteries,
redox flow batteries, and then metal-air batteries. The reasons for this will become apparent. We do also, in the chapter, consider other batteries. Lead-acid batteries have been used certainly for grid storage in the past and also high-temperature batteries. We'll just direct you to the study to read in more detail about those.
Now addressing the point of how much storage might be needed by mid-century. The round number that we'll use is 100TWh, this is arrived at through an analysis that is pretty granular. It's also supported by some other estimates that have recently been published. For instance, there's a McKenzie study that places the range of storage needed to decarbonize the electricity system by 2040 in the range of 80-140TWh. This is just for the electric grid it does not include electric transportation. Electrifying the light-duty fleet by 2050 could require another 144TWh, for example. This target of 100TWh by 2050, is one of the guideposts in what we did. Now,
before we get into the details of electrochemical storage, I want to explain the broader scope of storage technologies which you'll hear about in other webinars. This plot on the right has as its axis the power capacity cost or power costs, dollars per kilowatt. Then on the horizontal axis energy costs, dollars per kilowatt hour. What you notice here is the grouping of technologies. Lithium-ion batteries are typically made with relatively expensive materials. The storage of energy is costly, that's the horizontal axis, but it's able to deliver power at a relatively low cost.
It turns out that Redox flow batteries are somewhere in the middle and then Metal-air batteries as well as the other storage technologies that you'll hear about in other webinars, are on the left axis where the power cost is relatively high, but the energy costs meaning the materials that are used to store energy are exceptionally low. One looks at this and says, "Well, why can't we have it all and simply be at the origin of this plot?" That's certainly something we would all love to see, but it's constrained by physics and chemistry today. With this let me dive a little bit deeper and say something about why long duration and what does long duration mean. Today in much of the storage discussion, long duration means greater than four hours. There was a time when greater than two hours was considered long duration. Here we're really talking about multi-day gaps in generation
that need to be bridged, illustrated by this plot which shows in the black line, the load, and then the wind in the solar in the blue and orange colors across all 365 days in 2021. As you look at this what you see are the gaps between the renewable generation and the black line, the load, are gaps that extend over several days. That's why I say here multi-day storage. It's not pointing out seasons exactly and it's certainly more than a day so beyond the day multiple days of storage is working definition of long duration will mean here.
One of the requirements for bridging this gap is that, well, the competing technology or the incumbent technology is really natural gas generation. The next slide I want to present just a very simple way of looking at what is required of a storage technology in order to compete against natural gas on a cost basis. On the left side of this equation, we have the lifetime cost of natural gas plant, which I'll put it there $2,000/kW, and then if the duration that we're trying to bridge is multiple days, I'll choose a 100 hours, four days. What this simple arithmetic tells you is that the required battery cost, it has to be $20/kWh or less in order to compete with gas. When we compare that $20/kWh to where lithium-ion grid storage is today about $250/kWh becomes apparent why one needs lower-cost batteries for long-duration storage. With that in mind, and also noting that this required battery cost is for an installed system not just, for example, the cell or even the materials involved. The next plot here
which appears in our chapter is a way of looking at the cost of different battery chemistries. This is just the chemical costs which you see defined on the left-hand side here. Is the cost of the cathode, the anode, and the electrolyte divided by the energy stored which is the voltage capacity. This places a floor on the cost of the battery.
The battery cannot cost any less than the materials used to store energy. The vertical axis is a large scale and the horizontal axis is time, the date of introduction of that battery chemistry. On the left axis, you see the pre-1900 chemistries here. Nickel zinc, lead acid, and zinc air are all very early chemistries. Then you see approaching the current date, all these chemistries that have been introduced. If our goal is to get below $20/kWh, you see that well we have to start with something significantly less than 10 and that really narrows the available options here. We'll talk about these and I'll just even, at this
point, point out you see that the chemistries that use a metal or other low-cost inorganic and air as one of the electrodes for the lowest on this cost plot. We'll come back to these. Lithium-ion, the question whether or not lithium-ion given its incumbent status and can serve all storage functions is one that comes up often. Recognizing that lithium-ion battery prices have undergone this massive cost decline over about three decades now and that's the plot in the middle here. Back in 2000, we were above $1,000/kWh. Today we're down around 100 at the cell level slightly less. First of all, this cost decline is what has driven the massive expansion of lithium-ion in many applications. On the right-hand side is a plot that shows the manufacturing capacity of lithium-ion in GWh. The top of the scale is 2.5TWh. Over the current decade
2020 to 2030, there is a projected 20% compound annual growth rate in lithium-ion production, which at the end of the decade, so this is primarily driven by electric vehicle demand, at the end of the decade, we'll be at 2.5TWh of annual production capacity. When we compare that to the need for 100TWh by 2050, this implies that the compound annual growth rate would need to be sustained at greater than 20% for the next 28 years. That points out how heavy a lift it is to do all of grid storage with lithium-ion, even if the cost continues to decline we find lithium-ion chemistry is at a lower cost. Just as a passive in common the lowest cost lithium-ion storage for the grid that's on the horizon today is going to be lithium-ion phosphate based for several reasons, including cost and the material supply. Even in that case, however, the growth of production will need to be massive and sustained. This illustrates why we might want to look for alternative
chemistries. This plot is just a more granular look at the lithium-ion cell in this context and the stack bar chart here are all the cost components that go into a lithium-ion cell. The different vertical bars represent different lithium-ion chemistries from NCA, or nickel cobalt aluminum cathode to LFP, lithium iron phosphate cathode, and highlighting the parts of the bar are the electrolyte, anode, and cathode. If you add those up, you'll see that these all cases exceed $20/kWh, which was that long-duration cost target.
That illustrates some of the constraints of lithium-ion for multiday storage. The other one, which I'm only going to mention briefly here because the next hour we'll discuss this in much greater detail. These are results from the part of our chapter that Elsa already and Bob Jaffe led. This table shows you four of the elements that we would consider strategic to one case, lithium-ion chemistry and then vanadium, related vanadium redox batteries, which Fikile Brushett will talk about next. The third column over is the CAGR to reach 100TWh
by 2050. Highlighted in red are those cases where the CAGR and new growth rate needs to be greater than the historical CAGR, which is the first column, the column to the left. Then compare against that in the last column is the resource limit and highlighted in red there are cases where the resource limit may not allow you to reach 100TWh by 2050. This highlights the following point that it's in several of these cases, not the resource limit, not whether or not you have enough of that metal, but what the production of that metal needs to be in order to reach these storage targets by mid-century. Here, you see that, well, in the case of lithium, it's actually not the resource limit, but the fact that the production needs to be sustained at this very high level even though historically, there have been instances where lithium production has reached that level. Cobalt nickel vanadium, you see that you have to exceed the historical production increases in order to get there and you have to sustain those. This is the end of my discussion about lithium-ion
for grid storage. We believe that it will continue to be very widely used for short-duration storage, but the other chemistries will need to be developed for long-duration storage. With that, I'm going to now pass the mic as it were over to Fikile Brushett who will now tell you about redox flow batteries, and then I'll come back at the end and say more about metal-air batteries. Fikile, over to you. Fikile Brushett: Thank you, Yet. Good morning, everybody, at least this morning for me. I'm glad to be able to speak with you a little bit about maybe what might be some of the technologies that will complement lithium-ion batteries and will be useful for long-duration energy storage. I wanted to talk a little bit today about redox flow batteries. Yet, I'm going to have to ask you to click through a couple of animations here.
A redox flow battery essentially consists of tanks of solubilized energy storage material that are pumped through a reactor where they are charged and discharged. Yet, maybe if you could click to the next animation. Within the tanks, there is an active species that is dissolved in an electrolyte. The electrolyte, it can be something
like sulfuric acid and water. What you rely upon is the fact that the active species in each of these tanks have redox potentials that are different from one another. These disparate redox potentials gives you then the cell voltage. Yet, maybe you could click forward one more time.
This allows you to charge and to discharge the battery. One more click, Yet. Where are the reactions actually occur is in an electrochemical reactor. Yet, maybe click one more time, which is very similar to a fuel cell, a low-temperature fuel cell for those people who are familiar and essentially involves flow fields, carbon paper electrodes, and a membrane. The membrane, Yet click one more time, is particularly important here because it keeps the electrolytes apart from one another. What you want the membrane to do, Yet, click one more time is to allow ions to move back and forth between the two electrolytes to balance charge as the electrons move through the external circuit, but you want the membrane to block active species from going back and forth across. Yet, maybe click one more time. You want it to block the active species from going across, but allow ions to move through. You need permselectivity.
Abutted to that membrane or electrodes so-- Yet, if you could click one more time, and within these porous electrodes is where the electrochemical reactions occur. The active species is brought to these porous electrodes through engineered flow fields. The example that I'm showing you here is an interdigitated flow field, where the lighter blue areas are open channels and the darker areas are ribs. If you look at now the picture on the side there, those ribs then force the electrolyte through the porous electrode, where the electrochemical reactions will occur. Maybe, Yet, click one more time.
This force convection through these porous electrodes that are things like carbon paper lead to the redox reactions that occur on the surface of the porous electrode, and then the material will move out of the reactor and move back to the tank. Essentially, you're cycling these materials around and around to charge and discharge them when you want to store energy or when you want to release energy to the grid. Click, Yet, one more time. The potential benefit of this particular architecture for longer-duration energy storage is the decoupling of power and energy scaling. The energy is proportional to the tank size, or the number of tanks that you have. The power is proportional to the reactor size, or the number of reactors that you might have. You can independently specify these things and once you have an installation, it is possible to change these things independently to meet changing or emerging needs. The second is relatively simple manufacturing.
You're talking about mixing electrolytes, dissolving active species in acids or other sorts of electrolytes, and storing them in tanks. Then the reactors are relatively simple manufacturing, don't require clean rooms to do the assembly. They are relatively durable depending upon the active material. I'm going to come back to this, and require relatively low-cost maintenance. By the durability, what I mean is that the active materials are reacting on the surface of the electrode. There's no intercalation reaction, which could lead to materials decay. As long as your active materials are relatively stable, this system can run for a very long period of time. Then finally, there's relative location independence as compared to other long-duration potential storage systems such as pumped hydro storage or compressed air, or things of that nature. However, there's no free lunch and that's what this last click is here for. You're losing
energy density when you have a system like this as compared to lithium-ion because your active materials are dissolved in electrolyte and stored in external tanks rather than a compact cell as you'd have for a lithium-ion type battery. For this particular system, it looks more and more attractive, the longer and longer duration energy storage you have. As the tanks get larger, and the reactor gets smaller, you're asymptotically approaching the cost of the materials in the tank, the reactor becomes a smaller and smaller component. That
energy cost gets lower and lower until you get to the materials in the tank. If we go to the next slide, you might ask yourself, "Well, what's in the tank?" Vanadium redox flow batteries would be the state-of-the-art technology today. This slide shows the vanadium redox flow battery. If you look on the far left-hand side, you can see a simple diagram of the cell. What I want you to take away from this is that you have vanadium on both sides of the membrane. You're taking advantage of the fact that vanadium has four stable and soluble redox states within an aqueous water window, in this case, sulfuric acid. You have vanadium 23 on the negative side, the more negative side, and vanadium 45 on the more positive side. This is particularly
advantageous because the ion-selective membranes are not perfectly ion-selective. It's possible over long duration operation that some vanadium ions are going to move across that membrane and interact with the species on the wrong electrolyte. If you have all of the same species on either side, though, you're based upon the same parent compound rebalancing or resetting the system simply involves moving solution from one tank to the other periodically. This means that the system can be very durable and can run for a long period of time. Once it gets
out of balance, we can put it back into balance by shifting the electrolyte around. The second aspect here is that vanadium does not decay during operation. It does not turn into another species and so it can be recovered at the end of life and resold or replenished or it can be recycled. This also opens up opportunities for different economic models like electrolyte leasing, where you might lease the electrolyte from a company and the company might take it back after a while at the end of life or other types of recovery and re-utilization strategies towards the end of life. Vanadium flow batteries have been around since the 1980s. I think they were first
proposed by Professor Maria Skyllas-Kazacos, and have led to a number of companies working on them for many years. The example that I show in the middle is from Sumitomo Electric Company in Japan, but there's also companies in the United States, Largo Clean Energy in Massachusetts, and in Europe. The disadvantages of this particular system are the high cost of vanadium. Vanadium right now is quite expensive. You'll hear a little bit more about that in the next hour.
At least, initially, right now, these technologies are quite expensive as compared to lithium-ion batteries which hampers their ability to be deployed. In the long run, if we were thinking about using vanadium and we wanted to scale up and try to reduce the costs, what we might find is that we may run out of vanadium when we get to a certain amount of deployment. As we begin to think about these new technologies, the vanadium flow battery might carry us some of the way in terms of the flow battery technology, but it may not carry us all the way to multiple terawatt type deployment, and you'll much more about that coming up. If not vanadium, then what? If we go to the next slide, we might then think about, well, what electrolytes could we begin to think about using that are not vanadium related. What you find is that it's actually quite challenging to identify electrolytes that meet all of the criteria needed for low-cost efficient energy storage. We need to identify materials that have favorable redox potentials, those are the electrode reactions that are associated with the cell voltage, you want that to be high. You want the reaction kinetics
to be fast. You want them to be soluble in common electrolytes. You want them to be conductive and to work favorably with membranes. You don't want them to go across. They have to be inexpensive and they have to be safe. The point of this slide is simply to say that there are always
going to be trade-offs and compromises as you think about these new materials. Yet, if you go to the next click, there's always a trade-off for our compromise. As we begin to look for new materials, we want to look for effective property sets that allow us to get to low-cost safe energy storage. The last slide for me is the next slide to say, where are we going? There are two clear pathways that I can see towards lower-cost redox flow batteries. The first is on the left-hand side here is commodity scale inorganic materials, things like sulfur, iron. Materials that are abundant that have a materials production infrastructure ready,
and they may actually be used at the waste products that could be reused or recycled for long-duration energy storage. The challenge with an approach like this is the upgrading requirements, taking a material that's a waste product and converting it into an electrochemical product that might take some effort. There are also technical attributes that we need to be concerned about. These materials can't really be modified so they're going to
have a set efficiency and a set electrochemical reversibility that we'll need to work around, and this may lead to increasing cost in other system components that would be needed in order to realize these inexpensive energy storage systems. The second approach is to the right-hand side of the slide and that's engineered molecules via organic chemistry. The idea is, can we take organic molecules, aromatics let's say potentially from petrochemicals or from other feedstocks, and modify them through molecular functionalization to make them energy storage materials? This approach could be quite attractive because it would enable you to have tunable technical properties depending upon how you modify those reactive cores. They're based upon relatively abundant constituent elements and have the potential for mass production, at least for some of the targets, if we rely on ongoing organic materials production.
The challenge that those materials face is molecular stability. Can they last for as long as you need for long-duration energy storage in order to recoup your investment? There's potential cost and scalability challenges as the desired high-performing targets don't often match those materials that can be scaled at low cost. Identifying that nexus where we have materials to perform well enough to form long-duration energy storage, but are also cheap enough to be manufactured at scale is where we need to look for these types of materials. With that, I thank you for your attention. I'll be around for questions, but I wanted to pass it back to Yet. Yet-Ming: Thanks, Fikile. Let me now move to this third type of battery that we referred to earlier which is metal-air batteries.
Again, I'm showing this plot again just to show you down at the bottom, but what are our options in the area of metal-air batteries, and you see aluminum-air, iron-air, lithium-air. We won't talk about lithium or even really aluminum very much, but let me give you now an idea of what a metal-air battery is and what the issues are, what the advantages are, and why we think it's a category that has promised long duration storage. It turns out that almost any metal you can oxidize, you can make into a metal-air battery.
Especially, if it's a primary or disposable metal-air battery, and the zinc-air battery for hearing aids is the most common example. That is a battery where you, in the oxidation of zinc, you see the small image on the upper right here shows how this functions. You oxidize the metal, in the process you draw electrons, and then you produce an oxide or hydroxide which is a reaction product, and that then is how you discharge the battery. At the end of that process, you can throw it away or recycle it, but very few metal air chemistries are rechargeable. Lithium, zinc, and iron are the ones that have been demonstrated to be rechargeable although there are efforts to make others rechargeable.
In the periodic table here, you see some of the examples and also some of the companies who have worked on commercializing metal-air battery technology. Lithium-air has been primarily studied as a very high energy density battery chemistry for transportation reasons or applications. Rechargeable zinc-air has been studied by several companies and is one of the low-cost metals that one could conceivably use for rechargeable batteries for grid storage. There are ongoing
efforts as well as past efforts and in cases where thousands of batteries have been deployed in the field. The iron-air battery I'll spend more time on in the coming slides was originally studied by Westinghouse back in the 1960s. More recently, a company that I'm associated with, Form Energy has picked up on this chemistry and is developing iron-air batteries for grid storage.
Then finally, I'll mention that even carbon is something that can be oxidized. The carbon air battery is something that a company, Noon Energy, is working on commercializing. You see that there's a range of these, but not a very wide range. There are some clear
issues with metal-air batteries. One of the ones I'll point out right here is the fact that the round trip efficiencies are relatively low, but whereas a lithium-ion battery might have a round trip efficiency at low current rates of greater than 90%, here we're really talking about 40% to 60% route of proficiencies. Also, the balance of plant costs here that you require an air electrode, you require the supply of air, that you require management features that you don't need in the case of lithium-ion battery, so BOP costs tend to be higher. Now, the iron-air battery is one that, so using this as an example, this is one we might call just a reversible rustic of metallic iron. These two images show what happens during discharge
on the left-hand side. Discharge for a battery is always what is spontaneously downhill from an energy point of view. Energetically downhill, the iron wants to rust. The oxidation forming iron hydroxide is the discharge process, and the iron hydroxide is the discharge product.
The charge process simply refers to electrically recharging that battery, sorry unrusting that battery, and that's illustrated on the right. This can be done in an aqueous electrolyte environment using an alkaline electrolyte, which is relatively low cost. Now, it turns out that most versions of iron-air batteries actually use two air electrodes. If you think of the air electrode as the lung, this is an instance in which you have actually two sets of lungs, one for inhaling, one for exhaling. The air electrode for taking in oxygen, the oxygen reduction process, and making that oxygen available to the iron for oxidation, is referred to as the gas defusion electrode, or the ORR electrode, Oxygen Reduction Electrode. Then there's actually an evolution electrode which is the generation of oxygen gas that leaves the system. Then you have the third electrode which is metal anode. You see the reactions down here
at the bottom for the iron air case and on the right is an example of a charge-discharge process. No battery is perfect, all batteries have their flaws, and I pointed out the roundtrip efficiency. Let me explain just a little bit more detail where that comes from. The charge process you see here on the horizontal axis is the capacity. You see that in charging the battery more electrons go in, there's a higher charge capacity than discharge capacity. This is called a coulombic inefficiency. In this case, this comes from the fact that at the iron electrode the potential is very close to the hydrogen evolution potential for this system. You put some of the electrons
in charging into the generation of hydrogen, so that needs to be managed and that's one of the reasons for the round trip inefficiency, the coulombic inefficiency. The second is the gap you see on the voltage scale, the vertical scale between charge and discharge and that's a voltage inefficiency and that's primarily due to over potentials necessary at the air electrode. You see the limitations of the iron air battery, it's not a very good battery for a high-power rapid charge, rapid discharge scenario, not good for an electric vehicle, for example, but the very low cost is what makes it compelling. In designing an iron air battery for grid storage the question is whether or not they're very low cost, that advantage overcomes the other limitations. From a supply chain perspective,
iron is very interesting and what I show here is an image of the different states of iron as one goes from Iron Ore which is iron oxide, which is then pelletized. What I've outlined in the box in the middle here is the first stage in this iron supply chain where you have metallic iron. It's a material called Direct Reduced Iron or DRI which is actually some 95% metallic. At this stage, that iron oxide or iron ore has become reduced in this pellet form. This industry exists primarily to feed the electric arc furnace industry. To the right
you see some other forms of iron but the iron ore pellets here, DRI are used to feed EAF, Electric Arc Furnaces, which are a cleaner form of iron production and steel production-- I meant steel production, then the blast furnaces of the past. This is what Direct Reduced Iron looks like in volume. Here's a drum full of these pellets. On the right-hand side is an example of what a iron air battery, this is about 4 feet tall, 4 feet wide about a foot thick.
This is what a large-scale battery certainly, large scale compared to let's say your lead acid battery or your lithium-ion battery, what that might look like. There really is no supply chain limitation made here. These two images show the structure that you see is a vertical shaft furnace and there's a conveyor that brings the iron ore pellets to the top and over days they work their way down through this vertical shaft furnace and emerges that DRI that I showed you. On the left-hand side what you see those mounds in front of the
shaft furnace are big piles of iron ore pellets waiting to go through the shaft furnace. The numbers you see in the text here. If we were to reach a 100TWh even over just 10 years, it requires less than a 10% increase in global iron production and that's because there's such a large industry already in place for steel production. There's a supply chain in the US, there's a domestic supply chain as well as on every other continent on the planet. This is some of the reasons why low-cost metal-air, we believe, is one of the ways of meeting that less than $20/kWh greater than a 100TWh demand that might be required to decarbonize electricity system by 2050. Before I close with just some takeaways. I wanted to just show here in this slide some of the other technologies that you'll hear about, you've heard here about redox flow batteries of metal-air as well as lithium-ion. You will hear about
thermal energy storage in another presentation and also you'll hear about mechanical energy storage. There are some highlights here about what you'll hear about there. Very briefly in the thermal storage case, you'll hear about the challenge of heat to electricity and a particular approach that's been identified as low cost and relatively near-term. Which is to take existing
power plants, which already have the heat to electricity, and applying to the front end a thermal storage option for producing the steam. That is our collective view, the authors of this study an attractive option. You'll hear about mechanical energy storage, which is continuing to grow in other parts of the country, but it's really constrained in the US and elsewhere.
With that let me just close with this slide. Which is takeaways on electrochemical storage. Electrochemical technologies are the highest energy densities that storage technology that we have except for chemical energy storage. By that we mean hydrogen and that also it will be addressed in another presentation. On a power per area basis, these are attractive. The battery systems are typically relatively simple in their design, and they can be scaled. They can be applied at smaller scale as well as a larger scale. They scale down well there are a lot of storage technologies that do not scale down well. Just for something like residential storage,
for example, they would be more attractive. We discussed lithium-ion batteries. There is no apparent successor to lithium-ion in electric vehicle transportation today. Also, for short duration storage for the electric grid, today 4 hours or less in the future that may be 8 or 10 hours or less as the costs come down. We believe that they'll continue to be used in these short-duration grid storage applications.
One of the things that's benefited the deployment of lithium-ion for grid storage is the fact that they're used other applications, especially electric vehicles. The other technologies we've talked about redox flow management and metal-air do not have the benefit of drafting on another very large application such as electric vehicles. That's in some ways a constraint. However, the critical materials that need to be produced to take lithium-ion to a very large scale are running into supply constraints and you'll hear about that in the next presentation. This is why we end up with the main takeaway,
that for long-duration storage which we see is necessary. DOE as one agency should be supporting R&D and demonstration to advance longer duration technologies that rely on earth-abundant materials. With that, let me stop. I think we have about five minutes left for Q&A. I know that there have been a very large number of questions I see that have floated in as we've talked here. I'm going to stop sharing and let's see. Robert: Okay let me, put some questions to you,
Yet and Fikile. Before I do that, let me just point out to the audience if you would type in any questions into the Q&A button at the bottom of your Zoom screen. We'll try to get this many of these as we can. Secondly, a question that came up is with the recording of this be available and the answer is yes. We will send out a link to the recording in about two weeks as soon as we get it processed so that will be available. Let me start. One of the questions that came up, Yet and Fikile, is a question about the relative
lifetimes of these different chemistries. If you could speak to cycle life or over a lifetime. Yet-Ming: I'll just jump in. Fikile, can of course add. One things about long duration storage is that if you think about installations as being utility-scale and roughly 20 years of lifetime, the longer the duration the fewer the cycles you actually need. In some cases, in development of technology, recently there's been an emphasis on having many, many cycles, and then you realize that actually, you don't need that many cycles if you're doing multi-day storage and these full cycles. We can all do the arithmetic, and so the many thousand cycles that you're doing daily cycling over 10 or 20 years, but as you go to multi-day storage, over the entire lifetime of multi-day storage system, you might only see a few hundred cycles. That's actually a benefit for technology development. Fikile, perhaps you can say something about cycle life and calendar life for RFPs.
Fikile: Thanks, Yet. I agree with everything you said. The subtle difference to this question would be the type of decay mechanism that is impacting the battery. There are cycle-dependent decay mechanisms and there are time-dependent decay mechanisms. For cycle-dependent decay mechanisms, if you're not cycling as much, then it won't decay as much.
For time-dependent mechanisms, the more time you operate, it will decay more. In the case of flow batteries, just to put an example there, for many of the advanced organic or organic metallic or metal-centered coordination complex chemistries, their decay rates are dependent upon time rather than cycle rates. A big challenge in that area is identifying materials that are stable enough to either last for the duration of the installation or materials that are stable enough that periodic replacement becomes economically viable. Those are some of the things that we think about when we think about long-duration energy storage, at least within flow batteries. Robert: Thank you. One of the questions that came up, I'll just ask Kelly to provide a link to the future of energy storage study. Someone asked for a link to the report that Yet referred to. We'll send that out to the registered
attendees. Second question refers to the footprint, so not the cycle life, but the footprint of the different battery technologies, how much space do you take up, and does that impact where you might be able to use these batteries? For example, metal-air or redox flow batteries versus lithium-ion batteries, if you're trying to go that route. Yet-Ming: In comparison to lithium-ion batteries, lithium-ion batteries are very energy dense.
Both RFPs in metal-air batteries, they will take a more footprint, but compared to other storage technologies, they are still very energy-dense and very compact. For example, metal-air batteries their typical energy density fill the installation counting all of the balance of plant, would have a power per acre, which is roughly that of a natural gas power plant. That's a number of 1 to 2 megawatts per acre. In the context of the acreage required for wind or solar generation, that's going to be small. Unlike, for example, pumped hydro in which the footprint, of course, is going to be very large, for the same amount of power. Robert: Let me ask a very specific question about the solid-state batteries, question came up, what about those? Yet-Ming: That's a good question. Today, most of the emphasis has been on high energy density
and also high-value applications such as electric vehicles, high-value applications for solid-state batteries. That's where most of the R&D is. If the cost is there, grid storage would absolutely would make sense. I wouldn't rule it out, but I think I would put it in a similar category as lithium-ion today as being value for high energy density. At this point, at least not having yet demonstrated the kind of cost trajectory or the ability to use low-cost materials that would make it attractive for longer duration. This is coming from someone who spends a lot of time working on solid-state batteries.
Robert: Maybe one last question in this section. A number of people asked about these sodium-ion batteries and sodium-ion chemistry. Yet-Ming: I saw one of those questions. It is not yet at TRL6, it's one of the reasons we didn't include it. I think the short answer is that, yes, moving to sodium-ion for lithium-ion, there's a trade-off there in energy density and so the voltage of sodium-ion is typically less than that of lithium-ion. It's not a direct reduction in
costs just by substituting for lithium, but it certainly would help solve the lithium supply chain question. The manufacturing scale-up is similar, there still is a manufacturing constraint. Sodium-ion, I think, it's on the horizon. You'll just need sodium-ion gigafactories, instead of lithium-ion gigafactories. Robert: Fikile, any comments? Fikile: Yet said it well. Robert: Okay. Good. There are a lot of other good questions, some on environmental impacts and so on-- I think we'll bring those up during the next section where we talk about materials, but it's out to a great start. Thank you very much Yet and Fikile for those comments and answers to questions.
2022-08-08 23:41