Technologies for deep decarbonization using high temperature liquid metal
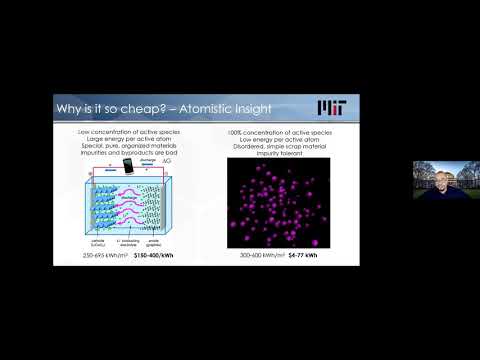
We’re very fortunate to have with us, Professor Ase Henry from Mechanical Engineering. Ase got his degrees in Mechanical Engineering Bachelors at Florida A&M University, Master’s and PhDs here at MIT in Mechanical Engineering. Then went to do postdocs at Oak Ridge National Labs in Northwestern University, and then spent a period of time at ARPA-E the Advanced Research Projects Agency of Energy at DOE identifying new program areas such as higher efficiency and lower cost energy capture, conversion and storage.
He began his academic career at Georgia Tech, where he was an assistant professor in Mechanical Engineering between 2012 and 2018. Then returned to MIT as an associate professor, where he holds tenure here in mechanical engineering. He’s made lots of advances in his young career so far. Including setting records for the highest temperature energy systems, including pumps that can work above 1,400° centigrade. For that, he actually holds the record in the Guinness Book of World Records.
Something that his children will probably know him for better than a lot of the details in his scientific publications. This platform he has for being able to manipulate high-temperature energy systems and to be able to scale those up through pumping and so on, has opened up a series of areas in grid-scale, thermal energy storage at very high temperatures, high-temperature pyrolysis of methane to get carbon-free hydrogen. He’s going to talk to us a little bit this morning about some of those methods and some of the opportunities it open up when you can actually work it at these very high temperatures.
I’m going to turn it over to Ase, and just before I do that let me remind you that you can submit questions through the Q and A, button at the bottom of your screen, and we’ll try to get to as many of those as we can following his presentation. Let me turn it over to you, Ase. -All right, thank you. Thanks, Bob. I appreciate the introduction. I’m planning to try to see if I can get to talk about three things today.
The first two will be about the work that we’re doing specifically in my lab. As Bob alluded to, we had a rather significant advance technological advance in my group pretty early on, which was this ability to pump and flow and control liquid metal at extreme temperatures. There are two technologies that we’re working on now that are outgrowths of that new capability. I’ll talk, talk about those in depth, so those will comprise the majority of it.
What I’ll also talk about at the end if I got time, I also want to talk about a paper we published recently that really just more generally, not specifically focused on high temperature with metal. More generally looks at what are some of the important opportunities for decarbonization, in general, for problems that involve thermal energy. I’ll try to run through what those are and give some insight into that. Now, the research that I do is actually- this stuff you’ll see today is maybe 60% or so of what I do. My bread and, butter is actually studying heat transfer at the atomic level studying the physics of heat transfer. That’s somewhat depicted in this image and other things that I do and some of these equations in the background, some new theory that we developed in the scope of studying the physics of heat transfer.
Some of that actually played a little bit of a role here in how it guided us towards the solutions that we are finding for technologies and the general approach that we tend to take. I’m not really going to talk about any of that work today, but maybe useful to know that this is not the full scope of everything I do. I actually do some basic science research as well. The first problem I want to talk about-- This is the paper that I mentioned recently. Just got put out in Nature Energy.
One of the key top five thermal problems that we talked about for decarbonization is actually thermal storage systems, and specifically this notion of energy storage in the context of grid-level storage. The way I see it, this is probably one of the most impactful single technologies that we could develop at this point and this is why I’m very much focused on it. If you look at electricity, it’s responsible for about 25% of greenhouse gas emissions. The transportation sector is responsible for about another 14% or so. If you add the two together, that’s about 40%.
Where does energy storage come in to play here? If you imagine what our most likely path is for very- really deep decarbonization, we have the best chance at trying to solve the energy storage problems, such that we can then decarbonize the entire grid. You can get the grid running on renewables. Then if you electrify the transportation sector-- Cars are now being run on electricity. That electricity is coming from the grid, that grid is decarbonized. You can, in essence, decarbonize these two large sectors with effectively one key technology that we don’t have today which is energy storage.
What is this energy storage problem? Well, over the last decade or so, the cost of solar and wind has decreased quite dramatically. So much so that in many parts of the world, solar and wind is actually cheaper on a purely levelized cost of energy basis. It’s cheaper than fossil. I say levelized cost of energy basis, that’s a key point, because that term or that figure does not actually account for when you get the energy.
When you have fossil energy, we get that energy whenever we want. We can dispatch it, we burn the fuel whenever we need the electricity. The supply is matched to demand as best we can as time goes on. In the case of PV and wind.
You only get the PV output when the sun’s out, you only get the wind output when the wind is blowing. Supply and demand are not synced up. This necessitates some form of storage. It’s also a problem for the grid if you were to-- This is a plot showing how much, for example, photovoltaic solar energy you’d end up throwing away as a function of how much flexibility there is on the grid.
If the system, meaning the entire grid, was powered at let’s say 20% PV energy and you only have about 60% flexibility, you’d end up effectively throwing away all of that PV energy. Meaning you have to have greater flexibility, which is a capability enabled by storage in order to reach higher penetrations where a large fraction or eventually the majority of the grid is actually powered by renewables. Storage is essential. Let me talk about this particular concept that we’re working on which is called Thermal Energy Grid Storage or the TEGS.
What we’re doing is we’re taking electricity, converting it to heat, storing it as heat, and then later converting it back to electricity. It’s electricity in from essentially any source agnostic to where it came from, you get electricity out. In essence, it’s basically like a battery. If you draw a dotted line around our stuff that we’re making for all intents and purposes, it takes in electricity, it spits out electricity.
Now, if you have ever taken a thermodynamic class on thermodynamics you would most likely think that this is a stupid idea, which is why I don’t think many people really thought about this very deeply until now. It looks like a bad idea, because if you look at how we get electricity today, we get electricity by converting heat to electricity, that’s how we get it. More than 90% of today’s electricity comes from burning some kind of fuel to make heat. Then that heat source then being used to make electricity.
In doing so, when we do that conversion from heat to electricity, we lose on average more than 60% of the energy. Most of the energy gets wasted. When I say wasted, that means it actually turns into wasted heat at a lower temperature. We end up throwing away most of the energy. This then begs the question, why would you ever want to pay this penalty twice? Meaning, we’ve paid it once to make the electricity in the first place. Now we’re talking about going backwards- going from electricity back to heat- only to store that heat and then pay the cost a second time to convert it to electricity and lose another roughly 50 to 60% or so of the energy.
Why would anybody do this? Well, the only reason you would ever do this is because storing the heat can actually be like 10 to 100 times cheaper than storing electricity. It’s cost that actually makes this all makes sense. We can be about 100% efficient, going from electricity back to heat. That we can do very efficiently. It’s this second conversion, going from heat back to electricity, that’s where you pay the penalty. That can never be 100%.
What we’re targeting in our technology is to get to about 50% efficiency conversion from heat back to electricity, but where we win is on the cost. Let’s talk through why would the cost be so cheap? This is where, as I alluded to earlier, my other work on the fundamental physics of heat comes into play a little bit in helping to give some insight into why would we ever even think of this. The reason is that when you store energy in different forms- and there’s different forms of energy- there are some intrinsic properties associated with storing energy in various ways. When you’re talking about a battery, you’re actually storing the energy electrochemically. There’s effectively a chemical reaction that would like to take place. Let’s say that this material here on the right wants to react with the material on the left.
You prevent that reaction by putting another material in between, and only allowing the atoms that are going to react to go through. In order to do so, they have to give up an electron and they give you electricity in order to go through this material. In order to set this situation up, what this requires is that you’ve got to get all these atoms arranged in this correct way on the right and on the left, and you’ve got to keep the atoms in the middle that are going to prevent the reaction from happening in an uncontrolled manner.
That all has to stay in place and operate the way that you want. If you do this approach, you get pretty great volumetric energy densities on the order of a couple of hundred kilowatt-hours per cubic meter. You can end up with costs in the range of hundreds of dollars per kilowatt-hour. There are reports or arguments that you may be able to get this down to $50 a kilowatt-hour.
However, some of the studies, more recent studies that I have looked at what costs we need to get to, to enable full penetration of renewables, is we have to get down to $10 or $5 per kilowatt-hour. Very, very low cost. Too low for batteries. Then why is thermal storage an opportunity? The reason that this can work or the reason that this makes sense on a fundamental level, is that unlike the battery over here, a very small percentage of the atoms in this battery are actually the ones participating and carrying the energy and are responsible for storing the energy. It’s a minority of the atoms. You have this whole scaffold. Most of the atoms that are sitting around they’re actually just being carried around to facilitate this reaction, but they don’t actually store the energy themselves. Conversely, with thermal energy storage, what we are storing the energy in is the kinetic energy of the atoms.
When you heat something up, the atoms speed up, their energy, their velocities increase, and they move faster, simple. It’s very, very tolerant to impurities. You can use very inexpensive, cheap materials that have impurities and things like that.
We don’t really care about the purity on this side. Purity matters a lot, because if you have impure elements or if you have impurities in this system, they can prevent this reaction from going in the way that you’d like. They can also cause side reactions. This can deteriorate over time. Generally, this has a shorter lifetime. But this process of essentially heating up atoms, making them hotter, and letting them cool down. Their kinetic energy goes up and down, up and down.
That cycle can happen many, many times without really any degradation mechanism. Essentially, all the atoms as they heat up and cool down are participating in the energy storage. It’s much less energy per atom as compared to a battery, but effectively all the atoms are participating.
This also gets you to a similar range of energy density, hundreds of kilowatt-hours per cubic meter, but the benefit is that the cost can be much lower. This can get down to just a few Dollars per kilowatt-hour. This is where we see the main benefit. If none of this makes sense, let’s walk through a simple estimate, just so I can walk you through, you can believe me when I say these numbers are this low.
This is hypothetical, imagine you wanted to store energy in liquid silicon. Again, the heat capacity is 950 Joules per kilogram per Kelvin. Cost of silicon- this is not semiconductor grade silicon, but metallurgical grade silicon- is about 58 kilograms. Silicon is one of the most abundant elements on the planet. It’s essentially the other part of sand.
Sand is basically silicon and oxygen. If you remove the oxygen, you get the silicon back. We have lots of that on the surface of the earth. It can be very cheap. Now, imagine you swing silicon through a temperature swing, meaning you heat it up by 500 degrees and then let it cool back down 500 degrees.
If you want to get the cost of that energy that you just stored, so to speak, in that process, that temperature swing, take the cost of the element, the cost of the material divided by the amount of energy in that temperature swing. It’s about 50 divided by CP delta T. This comes out to only $11 per kilowatt-hour. This is thermal energy, not electricity.
Now you have to pay an extra penalty, because you got to do this conversion back to electricity. Let’s say that that-- Believe me for a moment that we get to 50% efficiency, that’s a factor of two. That means your final cost of energy stored is more $20 per kilowatt-hour.
Now imagine, you go to something else even cheaper, like iron, like scrap steel. This cost is 10¢ a kilogram. Do the same 500-degree swing, and now you’re down at $3 a kilowatt-hour. This is the kind of materials and systems that we’re interested in, is getting to very, very low costs so that the cost is so low that even though we’re paying this 50% efficiency penalty, it’s actually worth it in the end. Now, one of the first things one might ask or raise their hand about, if you’re talking about storing heat is-- Everybody’s got this experience. You got your hot coffee or a hot tea, you put it in a mug, you put it on a table.
Come back in an hour, and it has cooled down. This is because heat is always going to leak from hot to cold. Even if you put it in a container and you insulate it, there’s always a finite amount of heat leakage from the hot stuff to the cold environment surrounding it. The issue though, is that the time it takes for something to cool down is actually a function of the volume to surface area ratio. If you make something very large, the amount of volume contained inside of it is very large compared to the amount of surface area available for all this heat leakage. The heat leakage scales with the surface area, the amount of energy that you’ve stored scales with the volume.
As you make something bigger and bigger, what happens is the time it takes for something to cool down, which is proportional to this ratio actually increases. If you’ve got a tank, and this is an example of a tank- molten salt tank for a concentrated solar power plant. There’s tens of thousands of tons of liquid salt inside here.
These are tanks on the order of 10 meters in diameter, on the order of 10 meters tall. On that order, you’re talking about a time cost, and the amount of time it takes just to cool down is months. All right, so long that this thing effectively stays the same temperature over the course of just a couple of days.
In those few days, you lose less than about a percent of the energy that you’ve got stored each day. This means that as long as you’re discharging your system, every couple of days, this leads to a small and arguably negligible penalty on the amount of energy that you’re losing. The key is that it does need to be big, and the bigger it gets, the better it gets. All right. This is one important aspect of thermal storage. The next question becomes well, what are you going to use? We talked about silicon, we talked about iron as possible storage materials.
One key piece that I’ll show you when we talk about how we move the heat around, and we’d love to do it with liquid metal, is that liquid metal is very corrosive to other metals. Maybe the best analogy is you can imagine, most metals will dissolve other metals. You can imagine, what if you tried to make a pipe for sugar water out of sugar.
Sugar water dissolves sugar. You can imagine that if you tried to flow liquid metal in a pipe made out of metal, you’ll dissolve away the pipe. It’s a similar arrangement.
One of our key insights here was that we realized that if you take certain liquid metals down in this range like Tin, Lead, Bismuth. These metals actually don’t like to bond with these other elements that are right above them. They don’t form very strong bonds with Carbon, Nitrogen, Oxygen or Boron. However, if you take one of these metals over here, these transition metals, bond them to one of these elements over here first, these form very, very strong compounds.
These are refractories. These are what we call ceramics. If you do that first, these materials actually have a very limited chemical interaction with these other materials. Why? Even though these metals would love to dissolve and bond with these, they’re not strong enough to break the bonds to these other atoms here. This is where a bit of the atomistic insight came from to really start looking at ceramics as a way to try to get to extremely high temperatures without corrosion.
Here’s a schematic of the basic idea of what it is we’re pursuing. The way it works again you store- take electricity, convert it to heat, store it as heat, and then convert it back to electricity later. The way this works is you take any electricity from wherever, doesn’t matter what kind of source. We use that electricity to run and power resistant heaters.
For all intents and purposes, you can think of this like just a light bulb filament, and we shine that light on some pipes. Those pipes heat up, and those pipes are containing a liquid metal. That liquid metal is being pumped. That liquid metals then flows through, carries that heat which is coming from the source of electricity that you want to store.
We convert that to heat, then store the heat in these large blocks. These are let’s say graphite blocks. One of the example that I told you about silicon, I told you about iron. It turns out another great option that has some great benefits, is actually to just store the energy in carbon and graphite. Graphite is like 50¢ a kilogram.
You pump the liquid metal over to these blocks, these blocks heat up. As they heat up, they are storing the energy that was the electricity that you wanted to store. These blocks then heat up to a peak temperature, and this is where the numbers start to sound crazy. The temperature swing that we’re looking at here is from about 1,900°C to 2,400°C. Again, about the temperature of a light bulb filament. The energy stays here in thermal form until you’re ready to use it again.
When you now want electricity again, you run your pump, extract the thermal energy from these blocks. They start to cool down and they provide you with hot liquid metal. Which you then run into this power conversion unit. The power conversion unit is a series of pipes. It’s like a heat exchanger, except that what these pipes are doing is they’re acting as a radiator. They’re supplying light.
One of the thing that’s different about this approach, instead of using a turbine like we would normally used to convert heat to electricity, we are instead using photovoltaics. One of the reasons we push the temperature so high to it’s extreme, is so that we can get these pipes glowing as hot as possible. Which shifts the spectrum of light that they’re emitting to the shorter wavelengths, to the higher energy so we can convert them very efficiently. These pipes are glowing white-hot, the whole infrastructure is glowing white-hot, and this light is hitting now PV cells, and these are not your normal PV cells.
These PV cells then convert the light back to electricity, and that’s how you get your electricity back. These PV cells are not normal. These are actually multi-junction PV cells.
We’ll talk a little bit about that in a second, but one of the most important things, is that these PV cells have a mirror on the back. The majority of the light, despite the fact that this is glowing white-hot, the majority of the light coming out of this infrastructure is actually still infrared. You can’t see it. The infrared light is actually not the light we’re most interested in. We actually don’t want to convert the low-frequency long wavelength light, we actually want to preserve that, so we use higher bandgap PV cells. We convert the light that we can convert very efficiently, the rest of it goes through the cell, hits the mirror, and then get sent back to the hot infrastructure where it’s been reabsorbed. In essence, we preserve the light that we can’t use or convert very efficiently.
This is where this moniker, "Sun in a box" came from. The idea is that we are trapping the light inside of a box. There’s insulation around here, so if you’re standing outside the system you’re not going to see anything glowing. All the glowing stuff is inside, it’s shielded. It’s like we’re trapping the sun inside of a box.
These PV cells are water-cooled, they stay cold. We can use a dry cooling unit, so we don’t see much of a penalty with them being up at like 35, 40°C. The other key piece that goes along with this that’s very exciting, is that these PV cells could be actuated in and out of the light.
You can imagine setting this up. This is like a cross section view of this unit cell here. You imagine that these PV cells could be mounted to actuators, and you can dip them into the light source to turn the system on and get output or you could retract them and very quickly reduce the output. The reason this is very attractive for grid scale storage, this will allow you to have gigawatt scale ramping on the order of seconds. Which currently doesn’t exist. What we do now is we turn on peaking turbines, and they take several minutes to an hour, for a turbine at full scale to ramp up from zero to full load.
This technology would have the ability to respond to grid needs much faster. It could do load following of PV output if a cloud goes by, and a particular PV field’s output drops. This kind of storage mechanism, this kind of storage approach could compensate very quickly. All right. Now one of the first things you’d also say is, you just talked about pumping liquid metal at 2,400°C, how are you going to pump it? This is the part that Bob alluded to very early on. This is the technological step that we made that preceded this, so we can pump it.
How we do that, is we can actually make all ceramic or all graphite pumps and valves and piping networks. The key piece here, the key innovation here is in the seals. Graphite is actually not a very expensive material to work with, it’s easy to machine. We do it in my lab all the time. The cost is in the range of a factor of two to steel.
It’s not much more expensive than steel, and you can make a hole graphite system very easily. This is what we do in the system that I showed on the previous slide. The system is envisioned to be made almost entirely out of graphite. Where our "Secret sauce" is really at the seals.
How do you join to graphite pipes so that you don’t get liquid metal leaking out. What you see in this video here, this is actually us pumping liquid metal for the first time, liquid Tin specifically. The same liquid metal we’re interested in using in our thermal storage system, and pumping it at about 1,400°C. This we can do with mechanical pumps, so actual moving parts that are glowing white hot. The materials actually can work under these conditions, and it was a big step. We have a paper showing it was a big step, that you could do this and not have these parts shatter or break under the loads that they experience in this context.
Another question one might ask is, why would you use photovoltaics instead of a turbine? You’re talking about 50% efficiency, and you could probably get maybe 60% with a turbine. The reason we’re focused on photovoltaics is a number of reasons. Number one, the turbine we’d want to use does not actually currently exists. It exists in people’s minds, but it’s never been actually made. This would have to be an external combustion turbine.
This would be a turbine that would require a heat exchanger to heat up the gas that’s going to go through it. These are components that don’t actually exist yet. We can write down on paper how they might be done, but no one’s ever made it to my knowledge. There’s a very large barrier to deploying a new turbine of this nature. You’re talking about 100 Million plus R and D effort. You’ve got to get buy-in, and you got to get GE or Siemens basically, ready to do this for you, because in an academic lab we’re not capable of developing a new commercial turbine.
You’d also need new materials, and heat exchangers, and again, you go back to this minute level or hour level response time to ramp up to full load. With multi-junction photovoltaics, we have a much lower barrier to deployment. There are companies that already make cells similar to this or can adapt their manufacturing equipment to make these kinds of cells.
The other thing is the cost can actually be considerably lower than that of a turbine. We can get down to like 10¢ a watt, rather than $1 a watt. We can get to almost the same efficiency range. I normally say 50% but our models actually suggest we can get above 50% to about 55 or so. We also get the fast response times on the order of seconds, and we can move down the cost curve much faster in this case. In the case of turbines, these turbines are well studied, well understood.
We know how low their cost could eventually get and it’s not sufficiently low to compete with this photovoltaics. However, with PV, if you come up with better PV cells after 10 years, you could go in and replace them, and also they may have lower maintenance. This is just a we have a paper and Journal Applied Physics. I’ll skip through this pretty quickly, because one question that often comes up is, why not keep it simple and just go single junction? In short, because we get a significant boost in performance from a second junction, so we’re going with dual junction.
This shows you for a choice of first bandgap material If you change the bottom bandgap material over this range. This is how the efficiency would change for different temperature ranges. Our nominal temperature is about 2,150°C. You can see here we can get to the mid in between 50 and 50% with a second junction versus down here with only a single junction.
It’s hard to get to 50% so the second Junction is important to get you a lot more current coming out of the cells. Just let me walk you through very quickly, incase you don’t believe me about this 50%. Let’s just walk through a quick energy balance. If you start with black body emission at 2,150°C.
2,150 is normally right between 1,900 and 2,400. You’d have almost 2 megawatts of light coming out per square meter. It’s very power-dense, but what we actually want to do is we actually don’t want carbon to do the emission. That’s another issue I can talk about, but in short, carbon’s vapor pressure’s too high and we’d like to actually have Tungsten facing our cold PV. We want to put a layer of Tungsten in front of it that prevents the carbon from getting to the PV cells. You take a significant hit though on the power density, because Tungsten is a reflective metal, so it actually does not admit as much light.
After the penalty you pay with Tungsten, here you get less than a megawatt, closer to 700 kilowatts per square meter, coming out of Tungsten. Now, like I said before, we are not even trying to convert the majority of the lights, so only this upper piece of about 200 kilowatts per square meter is the part that’s above the bandgap that we’re even attempting to convert. The rest of this light, the rest of this 476 kilowatts is below bandgap, we don’t try to convert it.
It hits the reflector and goes back. That reflector is not perfect, so if there’s a couple percent absorption, you get some heat absorption for the below bandgap light. Up here, we can convert those photons pretty efficiently, but not perfectly. You still get a bunch of heat generation for the current flowing in the cell, because you’ve got some inefficiency in the quantum efficiency. You’ve also got voltage loss. All these things accounted for, you end up with about 123 kilowatts per square meter of power output.
You end up with these other losses, there’s heat generation, there’s below bandgap absorption. We’ve also modeled the convection. Convection is small, but not negligible. That’s this other 4.6 kilowatts per square meter. You take the 123 divide by what you’re getting out.123 +
Q-gen + the below bandgap absorption and the convective losses. This still comes out to about 52%. We’re also expecting to lose less than a percent or so in the electronics for the heater, so you got to put on a lot of current to drive that heater. We’re also expecting to lose roughly a percent or so per day in heat leakage. There’s also on the order of a percent or less, parasitic heat leakage-- I’m sorry, parasitic power consumption, pumping the coolant around and keeping the cells clean. We expect in the end, we’re going to end up with about a 50% overall roundtrip efficiency after you account for all of these penalties.
Our 50% is what we think is real, 50% for roundtrip. The key thing is in the cost. Let’s talk about this graphite version I mentioned. How do we get below $10? The medium itself, the graphite is really cheap. It’s less than four bucks. You account for the installation, which is very significant cost.
That’s here as well. You’ve got the construction costs and other things. When you add them all up, it still comes out less than $10 a kilowatt hour, so this is very, very attractive. The total cost, however, is not just this cost of the energy storage, you take the cost per unit energy multiplied by the time, then you add the cost per unit power, CPP. The cost per unit power is down below about 40 cents a watt. This depends on how the PV cells are made.
We’re conservatively assuming the cost of the PV cells here, they’re being hit with lots of light. Again, it’s a much higher concentration of light, so to speak, that’s why the PV cost is as low as it is. There’s the inverter costs. That’s also very significant. Then there’s also the cost of running a dry cooling system. That’s also significant.
These things are all added in our cost models. Even still, with all this added, our costs come out lower and very competitive lower than pumped hydro. Where we’re at now, we have an RPE project that’s underway.
We’re trying to build a prototype, where we’ve actually demonstrated that we have Guinness-- On record with Guinness Book, having done 1,400C. We’ve actually pumped silicon at 2,000 CRA, so we’ve broken that record. We’ve got to apply for the new record. We’ve demonstrated running our heaters up to about 2,500C. We’re trying to deal with an issue of emitter evaporation, and deposition.
This is the point I made about Tungsten acting as a mass barrier for carbon deposition. We’re working on cell redesign and optimization and fabrication, got very high current density. There’s a lot of integration challenges with actually getting the current out of the cells.
Imagine a little square centimeter cell that’s got 10 amps coming out of it. You’ve got to touch it with some rather large wires. There’s a lot of integration challenges with doing that. We’re working on building a full-scale prototype that will fit inside this vacuum chamber. This is about a 10 foot-long, five foot in diameter vacuum chamber that we have in my lab. We keep the entire system in an argon environment.
With that, I’ll move on to the second technology that we’re developing, which is a methane pyrolysis reactor. This is a completely different problem, no longer talking about energy storage. If you think about hydrogen, the way we make hydrogen today is through a process called steam methane reforming.
Essentially two reactions. What you do is you take methane, which is carbon bonded to hydrogen, and you react it with water. Water’s got hydrogen in it and oxygen. You end up essentially exchanging the bonds. You want the oxygen from water to go with the carbon, so that you can make carbon dioxide first, and then later CO2.
What’s driving this reaction is the thermodynamic driving force to make CO2. That’s how you’re liberating the hydrogen. There’s really no way around this, other than making CO2. This CO2 production-- The hydrogen production produces about 1% to 2% of global CO2 emissions, so it’s significant. We’d like to do this a different way, which is called methane pyrolysis. This again, enabled by this new approach we have of pumping liquid at extreme temperatures, what we want to do instead is take methane, heat it up to about 1,400°C in the absence of oxygen.
We’re not combusting it. We’re heating it up and paralyzing it. At 1,400C, this molecule is no longer thermodynamically stable and it breaks apart and hydrogen gets liberated as gas, and then you end up with solid carbon. What’s different about this process on the left, is that you bring in gas and what comes out is gas. It’s all gases inside the reactor. This reactor, it’s very different, because you bring in gas and what comes out is solid and gas.
This presents a very important problem when you do direct thermal pyrolysis. If you bring in methane, the simplest thing you can imagine is, "Okay, I’ll put my methane in the tube. I’ll heat it up from the outside." What’s going to happen is you’ll get your methane to decompose. Hydrogen will come out the other end, but the carbon is going to deposit on the walls.
The problem with that is very quickly, it’ll block the entire cross-section of the reactor. You’ll plug your reactor. Now, the reason this is particularly bad is because it’s uneconomical to imagine running this in some batch reactor, where you heat up to 1,400C, flow for a little bit, make some carbon and hydrogen. Now you got to cool the whole thing down and waste all that heat, get a plunger and plug out the graphite or all the carbon that you’ve got, and then he ended up again.
That would be a very painful process, very uneconomical. One key thing with this is, how do you solve the plugging problem? How do you get rid of all this carbon that would block the reactor? We are not responsible for inventing this concept itself. There’s a very important contribution that we plan to make here. There’s a group in Germany that came up with a very clever idea of getting around this plugging problem. They solved the plugging problem. The idea interestingly, is to use liquid Tin.
Same liquid metal that we love to pump. Liquid Tin is peculiar in the sense that liquid Tin, although it’s in the same column of the periodic table as carbon, it actually forms no chemical compounds with carbon. There’s no Tin-Carbon phase diagram. It also does not form any compounds with hydrogen, so there’s no Tin hydrates.
Tin is inert with respect to carbon and hydrogen. What you can do is, you can take liquid metal Tin. Let’s say, you’ve got a big VAT of it at 1,400C, bubble up methane through it, and inside the methane bubble, the gas is going to heat up to 1,400C then the reaction is going to take place. It’s going to decompose, that bubble will now be filled with hydrogen and you’ll get carbon particles inside. Now, when that bubble reaches the top surface of the Tin, it’ll open up, the carbon will rest on the surface and the hydrogen will go out and you can pull the gas out separate.
Now that you’ve got this carbon floating on the Tin, there’s about a factor of two or so density difference between carbon and Tin. It’s about two and a half. The carbon will not sink into the Tin, and it’ll float. Because we can pump the liquid Tin, we can now use that as a mechanism to get the carbon out in a continuous manner.
We can flow the Tin and carry out the carbon with it floating on the surface. What’s illustrated here, and these are some of our innovations added to this, is that you can utilize like a ramp and have that ramp double as also a heat exchanger. You can cool the Tin and carbon down as it’s moving out. Do separations, separate out the carbon, pump the Tin back in and keep the Tin all in your system. You can also do innovative things like liquid droplet or direct contact heat exchangers, where you bring the liquid Tin directly into contact with this 1,400°C hydrogen, to actually do the recuperation and keep all the heat in the system. Here, this reaction of course, takes some energy.
It’s about 75 kilojoules per mol. This energy, we’re imagining has to come from some form of renewable resource, of course wind or solar. I can talk more about this later if anyone’s interested. What’s most exciting about this approach though, is the costs. Again come back to cost. What’s compelling here is the fact that when you do pyrolysis, you not only make hydrogen, you also make solid carbon and that solid carbon is actually valuable.
CO2 is like a pain, we want to pay to get rid of it. The solid carbon, we can actually use for something else. The general form of carbon that gets made in these reactors is carbon black. Carbon back sells from anywhere around $500 a ton up to $2,000 a ton depending upon its nano and microstructure. Even if we assume we’re at the very low end of the carbon black market at $500 a ton, this red line shows what our cost would be to sell the hydrogen.
This is where we would break even selling hydrogen. Our hydrogen cost could actually get below, not only the DOE targets but even below the current state of the art. We may actually be able to replace hydrogen production with a cheaper technology that actually makes carbon instead. The other key piece becomes wading into it all that carbon. I’ll show you in a couple of slides what we’re thinking of, but essentially we would like to solve, honestly, an even bigger CO2 problem which is to try to make a cement replacement.
Cement is responsible for about 10% of carbon emissions, CO2 emissions and we would like to make a construction material that’s almost entirely carbon that actually could replace cement. What’s shown on the right here is the respective percentages of the costs. The predominant costs here you have at buying your feedstock methane and the electricity, the reactor itself, the cost is not dominant. Again, this comes down to us being able to pump liquid tin.
What’s shown on the left here, this is a group in Germany. They’ve made a reactor before. Their reactor was made out of stainless steel.
Remember we talked about making flowing liquid metal or putting liquid metal in contact with another solid metal as your containing material. Liquid metals love to corrode or dissolve other metals and so their reactor had some issues because the tin started eating away at the steel. They couldn’t go very hot, the hotter you go the faster it happens.
Our main contribution is that we have this infrastructure. We can make an all-graphite infrastructure, an all-ceramic infrastructure and we can pump the liquid tin so we have no corrosion, no corrosion whatsoever at any time scale and we can go much hotter. We can go to 1,400 degrees C or hotter if we needed to. 1,400 degrees C is about where this reaction goes to completion, where you’ll get pure hydrogen, 99.99% pure hydrogen. This is just a glimpse of some of the components that we make.
For the components I showed like you have a cyclone, when you get to the colder temperatures below about 500 degrees C, tin melts at about 232 degrees C. Below 500, you can actually use stainless steel parts that are coated in ceramic. You can get away with ceramic coatings. This is showing suede lock parts that we’ve coated, for example, with titanium nitride. Titanium nitride is like the gold coating you’ll see on drill bits at home depot.
This material is ceramic and it doesn’t interact with liquid tin so you can protect standard steel components with a commercialized coating that exists already. These are old pumps and valves that we made that were actually all stainless steel, and we coated them with chromium nitride, and they work quite fine, no issues with any corrosion with liquid tin. When we want to go to the very high temperatures, we then switch to all-ceramic or graphite systems. This for example on the right here, this is an aluminum nitride pump. This was the first pump we made. We have essentially considerable experience now working on technologies in this temperature range.
This is an interesting problem that happens. This is a heater that we made, we use graphite. Resistant heater is one of the interesting things that happen.
You get this hot above 2,000C, and everything conducts electricity, including gas. One of the interesting things, problems, challenges that we had to solve is even making the heater gets interesting. You got to control and stay at low voltages which requires high currents, but what’s happening here is this heating element from the side view, we cut a hole in the insulation and the argon actually ignites and forms a plasma. What happens here is this the positive electrode, it actually arcs through the argon, goes through the insulation, and then comes back down on top of the negative electrode. That’s the shortest path, instead of going through this serpentine path.
We figured out ways around these issues but these are some of the important challenges that we’ve had to figure out. I’ll skip through most of this, we have a project that’s now funded by MIT to develop and demonstrate this reactor technology. We’re building a small prototype reactor inside my lab, again using these vacuum chambers to keep it in an argon environment.
We’ll be doing modeling, we’re also going to be building this cyclone to do the separations and demonstrate the separations as well. I’ll skip this, this basically shows the illustration, the principle for how we do the carbon extraction. You can imagine if you do nothing, the liquid level in these two containers will be equal. You turn on a pump, you can pump up the liquid level high enough, will go over this ledge and liquid tin will start pouring out on this ramp and then it will carry the carbon out.
This is the basic working principle for how we plan to extract the carbon. I had a collaborator at UCSD. As soon as we get some carbon out of our reactor, we plan to send it to him.
He has already made a construction material out of carbon. He can use carbon black that’s 95% or so carbon. He adds some binder and he can make something that’s stronger than concrete or steel-reinforced concrete. This is almost entirely carbon with some binder and he can make something stronger than steel-reinforced concrete.
I’m very interested to send him some of our material and actually show-- if he can show that he can make something in this range of strength out of the material that actually comes out of our reactor. That’ll help close the loop and show that the carbon that we would be making actually has this potential for functioning as a construction tool. This is just a quick glimpse of some of the things we’re doing now. We’re building a mock version of the reactor with plastic tubing and water to look at bubble flow and how we can control it. I’ll skip all that and very quickly just mention, I don’t think I have time to go through this but they’re essentially the top five problems in thermal science and engineering. We have a paper on this.
Thermal storage is one but you can see here on the right, if you look at global primary energy usage, almost all of it is heat. What we really want in the end for the majority is heat. We use all these various fuels, all of these various forms of energy that actually give us heat. If we look at how we get the heat, there’s maybe some other advances we could do that’ll help us save on carbon emissions. We talked about energy storage.
Another interesting aspect of energy storage is to use thermal storage on electric vehicles. About 35% to 40% of the battery capacity, of electrical battery capacity on an EV, can be used for space conditioning. You end up running your air conditioner and your heat sucking up about a third of the battery’s energy just trying to deliver heat.
Instead, you could actually just put a thermal battery on board that just does that portion of the energy and maybe more dense. That’s an interesting concept. There’s also this issue of we have to reinvent cooling. When you look at the global warming potential of the refrigerants we use, as it’s projected as you get big uptake in air conditioning units, particularly in Indian and developing nations, this could become 10% to 25% of global warming by 2050. We have to get off of this curve and figure out another way to do cooling that doesn’t have this problem. I mentioned cement, there are other problems like steel and aluminum, hydrogen that are also big contributors to CO2 emissions.
We’ve got to figure out ways of providing the heat that they need and reinventing those industries so that they actually are CO2 free. Another interesting problem is this issue of heating and cooling people. Generally, the way we do it is you got a building, you provide heating or cooling for the entire volume of the building, which is much greater than the volume of the people that you intend to heat or cool. If you can actually personalize heating or cooling and actually deliver it, similar to what we do with lighting, instead of lighting up the whole room, why not just put the light where you need it.
Instead of heating or cooling the entire room, why not just deliver the heating or cooling right where the people are, where they need it? There’s been some studies that have looked at this and shown and you get very very big energy savings if you could actually deliver localized heating and cooling, or zonal heating and cooling. Last interesting kind of far-out problem is, we’ve got an overabundance of waste heat right around the same temperature that we provide heating, but somehow we don’t have a wire to connect it. We’ve got all this waste heat from power plants at about 40 degrees C and then you want to provide heating for people at 40 degrees C. If we could find a way to develop a thermal wire, the equivalent of an electrical power line, but for heat rather than electricity, so that we can connect and send heat over long distances with little loss, this from a scientific perspective is an interesting question. There are some ideas for how to do this but this could help meet these residential and commercial loads with an overabundance of waste heat that we essentially reject to the atmosphere right now.
With that, I have a bunch of acknowledgments for where we’ve gotten our support and the students that have all played a critical role in this. I will be happy to take any questions that you have. -Great. Thanks very much, Asegun. It was a very interesting presentation, and generated lots of questions from the audience, as you can imagine. We have a little bit of time and I’d like to hit a few of those questions before we have to log off.
Let me start with a question about-- One of the challenges you pointed out earlier is that you have this inefficiency in going from thermal to electricity. If you get the electricity originally from a thermal process, of course, you’re going through that efficiency loss twice. What are your thoughts on integrating the original heat source directly with your thermal storage mechanism? For example, a lot of our thermal electricity today is in the form of coal or natural gas, and those are likely to go away. We almost surely will be left with things like nuclear, or hydrogen perhaps being burned in turbines. You would have the opportunity even in the net-zero future if we’re going directly from heat. What are your thoughts there? -Generally speaking, I expect our technology, this approach, to be most useful for renewables.
If you’ve got a fuel, put nuclear fuel to the side, nuclear fuel is special, because it’s really not like a fuel. You turn it on and you just use it up until you’re done and then you replace it. Fuels like hydrogen, even carbon-based fuels, they’re already fuels. They’re already storing the energy. You burn it on demand. There’s really no need to separately have storage, if you’ve already got the original form of energy in a storage form and you have a faucet, you can turn it up or turn it down. There’s not much of a need for storage under those conditions.
Where this is, I think particularly useful, is when you’re talking about renewables like solar and wind, where their input is not a faucet they get to control. The sun comes out, weather permitting, that’s when you get your output from PV, and when the wind is blowing is when you get your wind output. I think it could be integrated, but where I expect it would be most cost-effective is in the cases where you don’t already have a fuel. -Good. It might be worth thinking about whether
or not this could help with ramping, for example, you know, with the turbines that they don’t ramp as fast as you can do with MTPV. -Yes, definitely in that respect. Particularly when you think about the response time, you could probably extend the life of a turbine by taking some of the electricity off the grid with this kind of thing, to help balance in that sense. Yes, for sure. -A number of questions about the interesting materials problems that you raised and durability issues.
An example of the questions is, what would be the durability of graphite components? How do you seal these systems? A related seal question is, with MTPV, the mobile system, where you’re going to pull the PV in and out of some sort of a block to shield it, how do you do that motion? -Got it. Let me speak to the graphite issue first. We picked 2,400 degrees C for a reason. It happens to be related to this. Unlike metals and most of materials, graphite actually gets stronger the hotter it gets, up to about 2,500 degrees, and that’s why we stopped at 24. There is the issue of creep.
However, graphite’s creep resistance at 2,400 degrees C is sufficient for what it is where-- None of our parts are really under any load other than their own weight. The impeller for the pumps does not actually have to move that fast, so we can go at pretty low rotational speeds so we don’t have any particular creep issues there. The other key piece of this is that the power density is extremely high.
You actually don’t need very large pumps. Nominally, for a 100-megawatt system, you’re talking about pumps with a diameter of about a foot. You don’t really need large components that are going to experience significance and tropical stresses in that respect. The pressures are not very high.
10 meters or so, you’re talking about roughly speaking order 10 atmospheres of pressure. With regards to the seals, the way we seal, we have a couple of different sealing approaches, we use an all-carbon seal. There’s grafoil. Grafoil is essentially a number of products made out of it.
Basically, it’s a flexible form of graphite. That’s one of the key things you need if you want to seal something, if you want something squishy. These are seals that are already manufactured. We essentially figured out how to put them in an all-graphite system with carbon fiber composite bolts that have more strengths, so you can tighten down on them tight enough for them to seal well. There was this last question about the infrastructure for actually inserting and removing the PV.
We’re doing that now in my lab, we have a miniature version of it. You can imagine how it would look at scale. Basically, all we have is basically we have a long stick, some pipes. The pipes carry the coolant to keep the PV cold.
These things are mounted on a structure that’s on a rail system, with an actuator, so they can slide into, there’s a hole cut in the insulation, and the PV can slide right into the lighter, or you can retract it and pull it out. You just use a standard actuator to slide it in or slide it out, and you have flexible coolant hoses attached to it so that they can translate with it. At least in concept straight forward, we’ve already built these things. They work fine. I didn’t put any in new stuff that we’ve done in this presentation, but we’ve already demonstrated a lot of these things.
We’ve gone to 2,150C with the cavity and put the PV inside and things like that. These are the things that we’ve done. I’d like to show some results, maybe in an updated presentation a year from now, so we can actually show some really high efficiencies. -If you have another minute or two that we run over just a minute and ask a few questions about the hydrogen production case that you showed. -Sure.
-Question there is, how does the cost or performance of the pyrolysis system compare with electrolysis of water to make hydrogen? -In short, I don’t know the cost of electrolysis off top. My understanding though, is that electrolysis has not yet beaten this DOE target of $2 a kilogram. We are expecting to get significantly below the DOE target, and actually to a place that we actually can compete with the current state-of-the-art, which is around here, just under about $1.50 a kilograms. -A related question was, looking at the economics you show here, when you think about going to scale, do you think of this as a hydrogen production process or a carbon black production process? -Good question. I’ll say, if you’re doing it right,
basically your main revenue source is the carbon. This is really a carbon black production facility that happens to put out hydrogen. There’s actually a company that’s doing this now, not this design, not this particular approach, but they do do methane pyrolysis just to sell carbon and actually throw their hydrogen away. It’s called Monolith Materials. They do a very different process.
They try to make the highest-value carbon black possible. They’re closer out on the $2,000 per-ton scale in terms of the cost they’re able to get, or let me say, the price they’re able to get for their carbon. They don’t even worry about the hydrogen. Where I’m interested in is more so having an impact on climate change. I’m interested in how we can actually make this reactor system, and can we get it small enough to fit in a shipping container? If we can keep it small and make it economically viable when it’s small, I’m really interested in-- Of course, you can make it a very large one that’s very efficient and cost-effective, but if you can make a small one that also does that, there are a lot of stranded natural gas sources far out in the oil fields that I think that would be really interesting to drive a shipping container out there, convert it to hydrogen, and then send out a dump truck every week to take in a couple of tons of the carbon that you make. That would help cut down on emissions as well, and would provide a local source of hydrogen that could be used.
-Related to that idea of a small modular system you could take out into the field, there was a question about scaling, and the demand on tin production. If in a realistic system you’ll probably lose a little bit of tin to the carbon black, as you’re doing the separation, so you’d have to make that up. If you’re trying to go at scale and replace some SMR for production of hydrogen, or even SMR plus CCS, with this pyrolysis idea, what would be the implications for tin supply? -Great question. In short, I don’t remember the number off top, but we did look at this. It’s minuscule. The big usage of tin today is for solder and basically, the best price you can get for tin is about $2 a pound and that’s buying hundreds of tons per month. This kind of reactor wouldn’t use nowhere near that amount, it would be maybe pounds per month, not thousands of pounds per month.
It would pale in comparison to the primary usage of tin, which is for solder. There’s a couple interesting issues with this issue of loss of tin through the separations. You’re absolutely correct, there’s going to be some finite amount of tin that will end up in your final product for the carbon. The cool thing, though, is once you get it cold and the tin freezes, now you can do solid to solid separations. You can make another cycle and do even better separations and get the tin out as well and possibly further suppress this need to do makeup tin. My bigger concern with makeup tin is actually impurities in the incoming method.
If you’ve got some residual oxygen, other kinds of hydrocarbons I don’t think will matter once you get to the 1,400 C they’re all going to decompose, but I’m more concerned about sulfur and some residual oxygen in the methane strip. Those are things that could bond to tin. You can make some tin-oxide, that would be the main thing that would eat up some of the tin.
-Thank you very much, Asegun. This is a fascinating presentation. You’ve obviously captured the interest and imagination of the audience. We have lots of questions and unfortunately, we just can’t get to them all. Thank you for the stimulating presentation, and thanks for that buried offer in the presentation to do an update on this a little while from now.
I think it’ll be great to see where this goes. It’s very promising. -Anyone who’s got a question, if we didn’t get to it today, please feel free to send me an email. -Good. We’ll post this recording on the MIT website so the people who had to log off early can capture some of this.
Thank you so much. I look forward to seeing you back on campus in the new year sometime. Thank you again. -Thank you. -Goodbye.
-Take care.
2020-12-19 07:34